#material science
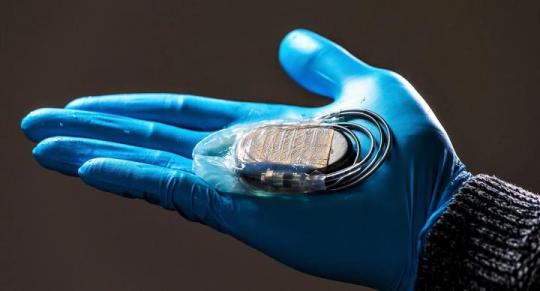
Above: A cellulose membrane for protecting pacemakers. Image credit: Hylomorph
By Anthony Caggiano
A cellulose membrane that can reduce the buildup of fibrotic tissue around cardiac pacemakers has been developed by ETH Zurich, Switzerland, scientists.
It could be used to help ease surgery when a pacemaker needs to be replaced.
Aldo Ferrari, a Senior Scientist in ETH Professor Dimos Poulikakos’s group and at research institute Empa, said if too much tissue has grown around a pacemaker – which usually has a lifespan of five years when its battery expires – it can be hard to remove the device in surgery. The surgeon may need to spend time cutting the tissue, and risk of infection may increase.
Ferrari and his colleagues at ETH Zurich have developed a membrane with a special surface structure that is less conducive to the growth of fibrotic tissue than the smooth metal surface of pacemakers.
This membrane has now been patented and Ferrari is working with fellow researchers at the Wyss Zurich research center, the University of Zurich and the German Center of Cardiovascular Research in Berlin to make it market-ready for use in patients.
To test the device, pigs were given two pacemakers - one enveloped in the cellulose membrane, one without. After a year of testing, the researchers found the pigs’ bodies did not reject the cellulose membrane.
‘This is an important finding because tolerance is a core requirement for implant materials,’ Ferrari said.
The fibrotic tissue that formed around the membrane was about a third of what grew around the unencapsulated pacemakers.
Two reasons have been identified as to why this may have happened. The first is because the material is fibrous by nature. Francesco Robotti, lead author of the study and a scientist in Professor Poulikakos’s group, explained further.
'When fibrotic tissue forms, the first stage is the deposition of proteins on the surface. A fibrous membrane surface impedes this process,’ he said.
The second factor is the researchers created the membrane with honeycomb-like indentations in the surface, each measuring 10 micrometres in diameter.
'These indentations make it difficult for the cells that form fibrotic tissue to adhere to the surface - the second stage in the formation processes,’ Robotti said.
The scientists want to apply for approval for clinical trials in humans in partnership with ETH spin-off Hylomorph, which will be responsible for commercialising the membrane. The trials are slated to start next year at three cardiac centres in Germany.
The findings were reported in Biomaterials.
7.9.2019.
Spent the morning before my lecture to create phase diagrams for one of my master’s projects. Trying to estimate the solid solution strengthening possibilities of all the elements in a system.
Post link
Aluminum target for our sputter system. If you look closely you can see individual crystal grains.
Sputtering is a technique used to deposit thin films of material. The material source is called a target because it is bombarded with high energy atoms which remove bits of material that are then redeposited on your sample or wafer. The ring is a result of the magnetic field confining the plasma to that region.
Post link
Discarded AFM tips.
Atomic Force Microscopy, or AFM, is a technique by which a small mechanical probe is scanned across a sample to create a height map. This technique has very high resolution, less than a nanometer, depending on what kind of tip is being used, and can be done in ambient conditions (no need for vacuum). AFM is useful for getting roughness data and measuring film thickness, and can be combined with other microscopy techniques to get a complete picture of your device.
AFM probes often get damaged or dirty, resulting in “tip graveyards” like the one shown here.
Post link
Sample stage controls for an ion mill, allowing for rotation about two different axes.
Ion milling is a type of dry etch process used to remove parts of a sample by bombarding it with ions, typically argon, in a vacuum chamber. It can be thought of as an atomic sand blaster. Ions (the “grains of sand”) physically expel, or sputter, chunks of material from the surface. The sample is rotated to ensure uniform coverage.
Post link
Patterned silicon wafers in the clean room.
Photolithography is a technique similar to photography used to make very small micron (~0.00004 inch) sized features. Wafers are coated in light sensitive chemicals called photoresist and then certain areas are exposed to light to create a pattern. The processing room is lit with yellow light to avoid exposing the resist. Photolithography is commonly used to make integrated circuits in electronics, but it is also used in basic research in fields such as physics, material science, engineering, and even biology.
Post link
Cryopump on a sputter system.
Cryopumps are a common type of high vacuum pump. They operate by condensing or freezing gases in the vacuum chamber onto an array that is typically cooled down to 10-12 kelvin. Compressed helium gas enters a chamber below the condenser array, where a piston (the displacer) allows the gas to expand, removing heat from the system. The piston then pushes the helium out and back into a compressor, and the cycle repeats. The displacer and compressor in a cryopump make a distinctive rhythmic high pitched whistle that is a common sound in clean rooms and condensed matter physics labs.
Post link
A sputter system in our lab.
Sputter deposition is a fabrication technique used to deposit thin films of a particular material onto a sample. The film can then be patterned using lithography into, for example, electrical contacts for your device. It is commonly used in the semiconductor industry to make integrated circuits.
First a gaseous plasma of ions, typically argon, is created in the sputter chamber. Ions in the plasma are then accelerated into a large piece of the material to be deposited, called the target, causing atoms to be ejected from the surface. Atoms that reach the sample or substrate are redeposited, forming a thin film over time.
Post link
Platinum plasma in our sputter system.
Plasma is the fourth state of matter (in addition to solids, liquids, and gases) that can be best described as an ionized gas. It is a high energy state, in which there is sufficient energy to strip electrons from atoms or molecules in a gas. The plasma glows because when the electrons recombine with atoms, energy is released in the form of light. The color of the light depends on the composition of the plasma. The plasma in this picture is purple mainly because of the argon ions used to bombard the platinum.
Surprisingly, even though plasma is probably one of the least known of the states of matter, it is the most common in the universe. Examples of plasmas encountered in nature include lightning, some types of flames, nebulae, and stars.
Post link

Authored by Kenny Walter, Digital Reporter, R&D Magazine
Engineers have created new computer maps that could help design platelet-matrix composites that replicate some of nature’s toughest substances.
Researchers from Rice University have developed a method to decode the interactions between materials and the structures they form, which could help maximize their strength, toughness, stiffness and fracture strain.
Read more: https://www.rdmag.com/article/2017/12/scientists-mimic-natures-toughest-substances
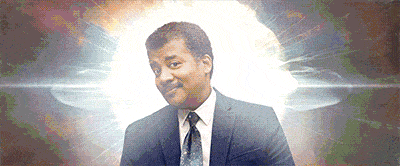
1. Scientists just used super-computers to predict and design two new types of magnetic materials
2. Replacing traditional antibiotics with a CRISPR-pill is the future for hospitals and nursing facilities
3. Hydrothermal vents with hydrogen on Saturn’s moon could hold life (those vents are basically where life began here on Earth)
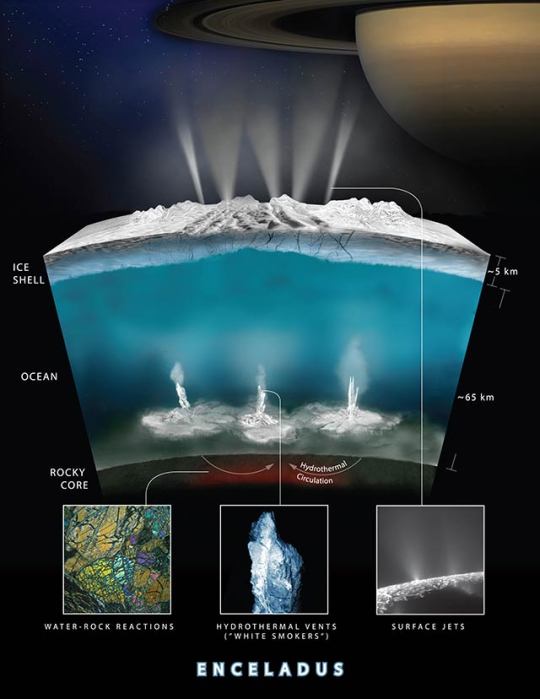
4. Self-taught AI is slightly better at predicting heart attacks than your doctor
5. Your shoe-laces actually come undone in one or two strides (rather than slowly throughout the day)
6. This device can pull nearly 3 Liters of water from dry air in 12 hours
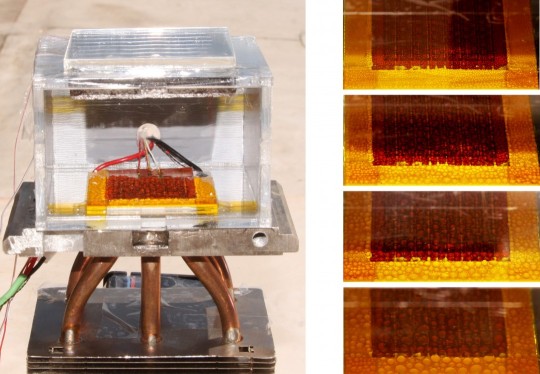
7. The March for Science is on the 22nd, many are concerned the march will fail to be non-partisan enough
The Science March’s twitter backtracking on some identity politics
8. Also NASA is putting the Earth up for adoption for Earth Day
9. A black hole simulation in 1978 looks just like the supercomputer simulation used in the movie Interstellar
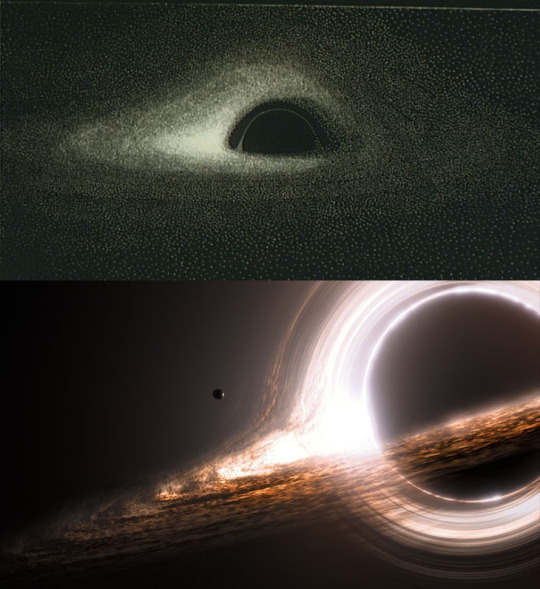
10. Behold the horrifying mess that is your adult teeth ramming their way through your skull
11. Finding the one true caveman diet is ridiculous because humans lived essentially in all climates and food sources evolved throughout history
12. Archer fish are actually just nature’s bidet for insects
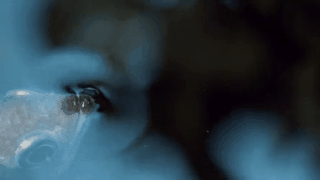
13. More evidence that shows high-energy events like lightning strikes or meteor impacts can create building-blocks for life
14. Here’s what an earthquake looks like under water
15. Scientists design a special drone to study volcanoes
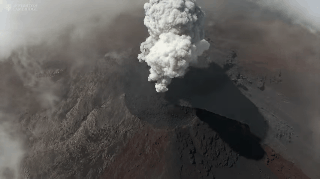
16. For your weekly dose of pseudoscience bull-shit: Naturopath kills patient with IV Turmeric
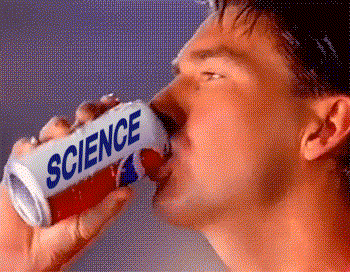
5 Amazing Scientific Discoveries We Don’t Know What to Do With
Every day, scientists make discoveries that change the way we live. But sometimes, just sometimes, they achieve results that are so extraordinary or unexpected that they literally don’t know what to do with them. Here are five of the most puzzling.
Mind-bending material properties
In January, a team of physicists from Rutgers and MIT published a paper in Nature describing a new property of matter. While fiddling around with a super-cooled Uranium compound, URu2Si2, they found that it breaks something called double time-reversal symmetry. Normal time-reversal symmetry states that the motion of particles looks the same running back and forth in time: magnets break that, though, because if you reverse time, the magnetic field they produce reverses direction. You have to reverse time twice to get them back to their original state.
This new material, though, breaks double time-reversal symmetry. That means you need to reverse time four times for the behaviour to get back to its original state. It’s something the scientists have dubbed hastatic order - and if you’re struggling to get your head round it, well, that’s the appropriate reaction. The scientists who discovered the phenomenon can’t explain a good physical example of what it is, how it works, or what it means.
The universe weighs less than we thought
When the world’s best scientists decided to team up and measure the mass of the universe all the way back in the 1970s, they set themselves a pretty tall challenge. Applying their best understanding of gravity and the dynamics of galaxies, though, they came up with an answer - an answer which sadly predicts our universe should be falling apart. We know that the Universe’s matter orbits a single central point and that must mean its own motion generates enough centripetal force to make that happen.
But calculations suggest that there’s not actually enough mass in the galaxies to produce the forces required to keep it moving in the way we’ve observed. So physicists scratched their heads, worried a little, then proudly stated that there must be more stuff out there than we can see. That’s the theory behind what everyone now refers to as Dark Matter. The only problem? In the past 40 years, nobody has confirmed whether it really exists or not - so, effectively, the problem thrown up by those initial calculations remains.
The placebo effect
Feed a sick man a dummy pill that he thinks will cure him and, often, his health will improve in a similar way to someone taking real drugs. In other words, a bunch of nothing can improve your health. In theory, it could be a poweful treatment technique.
But experiments have shown that the kind of nothing you deliver matters: when palcebos are laced with a drug that blocks the effects of morphine, for instance, the effect vanishes. While that proves that the placebo effect is somehow biochemical (and not just a psychological effect) we know practically nothing else about the power of placebo.
It’s real, sure. It can help people get better, agreed. But if we’re ever to make anything of the much-studied but little-understood effect, we’re going to have to unpick how the mind can affect the body’s biochemistry - and, right now, nobody knows.
Temperatures below absolute zero
It used to be that scientists all agreed that it was impossible to achieve temperatures below absolute zero. It was literally the coldest anything could ever get. Late last year, though, a team of scientists from the Max-Planck-Institute in Germany blew that out of the water: finally, they’d cooled a cloud of gas atoms to below −273.15°C. In fact, the result was as much a quirk of the definition of temperature as anything else, and the way it relies on both energy and entropy (the measure of disorder of particles). New Scientist explains:
In principle [it’s] possible to keep heating the particles up, while driving their entropy down. Because this breaks the energy-entropy correlation, it marks the start of the negative temperature scale, where the distribution of energies is reversed – instead of most particles having a low energy and a few having a high, most have a high energy and just a few have a low energy.
It’s this curious logic that allowed the Max-Planck-Institute researchers to cool a variety of atoms in a vacuum, for the the first time ever, to below absolute zero. So far, though, they haven’t managed to work out what to do with the chilled particles.
Cold fusion
Back in 1989, a pair of scientists -Fleischmann and Pons- claimed that they’d achieved a remarkable feat: they’d successfully observed nuclear fusion at room temperatures. Momentarily, the finding was heralded as a revolutinary discovery that could transform energy production around the globe. Sadly their experiments weren’t reproducible, but they did inspire scientists to study cold fusion in more depth.
Turns out, the process is in fact theoretically possible. For two atoms to fuse together, they need to come close enough to each other to overcome their mutual electric repulsion, which is caused by the cloud of electrons that orbit them. Usually that’s made possible by super-high temperatures -like at the center of the sun- but quantum physics suggests that there is at least the possibility that atoms can fuse without the need for energy injection via high tempeatures.
And it’s that hope that means a small band of scientists still work in the shadows, trying to get cold fusion to work. Of course, while occasional results come and go, they tend to be rather dubious. Fundamentally that’s because, even though quantum theory tells us it should be possible, nobody knows how to use that understanding to actually get a fusion reaction going.
The Higgs Boson
Just kiddin’. We’ve known what to do with Higgs since forever.
Post link
Let’s look in detail at the process of boiling a pure substance.
We’ll start out with a compressed liquid. We’ll say it’s water at 25 deg C. Moreover, let’s say it’s contained in a cylinder with a piston so that the pressure inside the cylinder is always at atmospheric pressure.
If we start heating up the cylinder, the water inside will expand slightly. The pressure will remain constant as the piston moves with the water’s expansion. Once we get to 100 deg C, the water exists as a saturated liquid - any additional heat will cause it to vaporize.
If we keep adding heat to it, things get interesting. The water will start to boil. It’s volume will increase drastically, but its temperature will remain the same. All the energy you’re putting into it is going into the phase change. The amount of energy it takes to go from a liquid to a vapor is called the latent heat of vaporization. (Similarly, the amount of heat it takes for a solid to melt into a liquid is the latent heat of fusion.) So while the water in your cylinder is in the process of boiling, it exists as a mixture of saturated liquid and saturated vapor. Its temperature will remain at a constant 100 deg C throughout the process. Although its apparent volume will increase, its specific volume - the volume per unit mass - will also remain constant.
Once all the water has been vaporized, if you continue adding heat to the cylinder, the water will start to rise in temperature again and its specific volume will start to increase. In this state, it exists as a superheated vapor.
The entire process we just described looks like this.
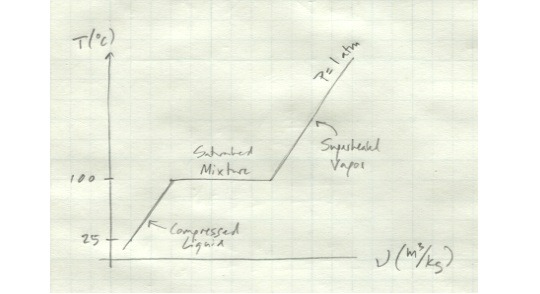
Note that this show temperature vs. specific volume for only one pressure - if we varied the pressure as well, things would look quite different. The interdependence of temperature, volume, and pressure will be important in our analysis of thermodynamic processes.
We’ve spent some time going over the basics of heat enginesandrefrigerators. These are machines which manipulate a working fluid through some sort of cycle in order to move heat from one location to another. We have some idea of the overall way they work, but to really understand the details of what’s going on, we’re going to have to know a little more about how this working fluid behaves.
For right now, we’ll restrict our discussion to materials which qualify as pure substances. A pure substance has the same chemical composition throughout - all its molecules are the same. We’re all familiar with phases of matter - solid, liquid, and gas are the ones we see on a daily basis. Matter in solid form has its molecules arranged in a regularly structured lattice. In a liquid, molecules have about the same distance from each other as they do in a solid, but they’re not held in a structure and can move around each other freely, although their close spacing means that intermolecular forces play a large part in their motion. In a gas, molecules are widely spaced and careen about as they please, interacting with each other only through collisions.
For thermodynamics-related purposes, we’re really interested most in liquids and gases. Controlling the transition between these two states is the key to moving a lot of energy around. So let’s define some terms.
Acompressed liquidorsubcooled liquid is one that is in no danger of vaporizing.
Asaturated liquid is one that is about to vaporize.
Asaturated vapor is one that is about to condense.
Asuperheated vapor is one that is not in any danger of condensing.
Distinguishing between these is important from a thermodynamics because a fluid will have different properties related to its ability to absorb and release energy in each state. In coming articles, we’ll look a little more closely at the exact process of transition between phases.
materialsscienceandengineering:
Upsizing nanostructures into light, flexible 3-D printed metallic materials
For years, scientists and engineers have synthesized materials at the nanoscale level to take advantage of their mechanical, optical, and energy properties, but efforts to scale these materials to larger sizes have resulted in diminished performance and structural integrity.
Now, researchers led by Xiaoyu “Rayne” Zheng, an assistant professor of mechanical engineering at Virginia Tech have published a study in the journal Nature Materials that describes a new process to create lightweight, strong and super elastic 3-D printed metallic nanostructured materials with unprecedented scalability, a full seven orders of magnitude control of arbitrary 3-D architectures.
Strikingly, these multiscale metallic materials have displayed super elasticity because of their designed hierarchical 3-D architectural arrangement and nanoscale hollow tubes, resulting in more than a 400 percent increase of tensile elasticity over conventional lightweight metals and ceramic foams.
Post link
